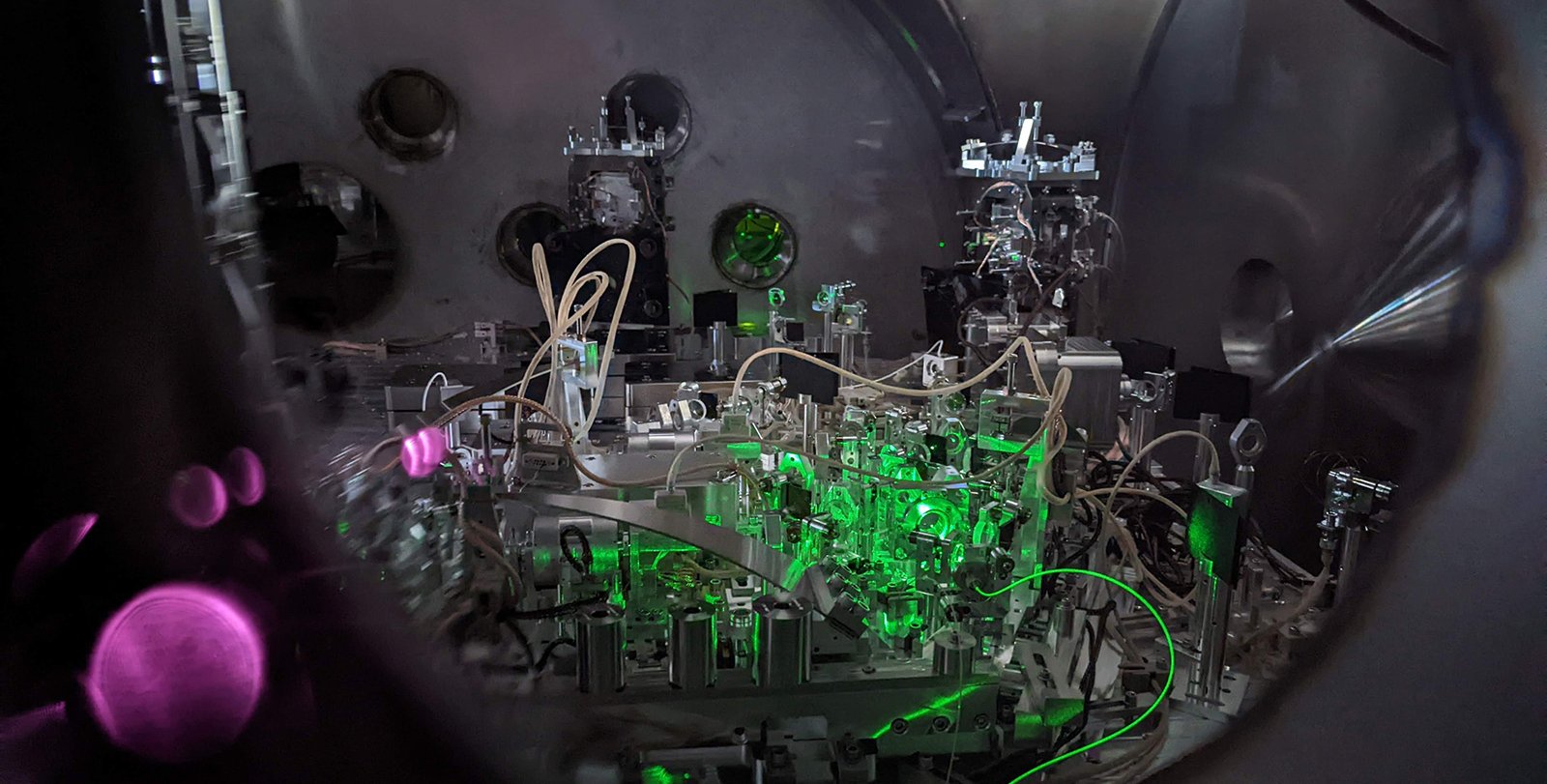
LIGO Surpasses the Quantum Limit
In 2015, the Laser Interferometer Gravitational-Wave Observatory, or LIGO, made history when it made the first direct detection of gravitational waves, or ripples in space and time, produced by a pair of colliding black holes. Since then, the U.S. National Science Foundation (NSF)-funded LIGO and its sister detector in Europe, Virgo, have detected gravitational waves from dozens of mergers between black holes as well as from collisions between a related class of stellar remnants called neutron stars. At the heart of LIGO's success is its ability to measure the stretching and squeezing of the fabric of spacetime on scales 10 thousand trillion times smaller than a human hair.
As incomprehensibly small as these measurements are, LIGO's precision has continued to be limited by the laws of quantum physics. At very tiny, subatomic scales, empty space is filled with a faint crackling of quantum noise, which interferes with LIGO's measurements and restricts how sensitive the observatory can be. Now, writing in the journal Physical Review X, LIGO researchers report a significant advance in a quantum technology called "squeezing" that allows them to skirt around this limit and measure undulations in spacetime across the entire range of gravitational frequencies detected by LIGO.
This new "frequency-dependent squeezing" technology, in operation at LIGO since it turned back on in May of this year, means that the detectors can now probe a larger volume of the universe and are expected to detect about 60 percent more mergers than before. This greatly boosts LIGO's ability to study the exotic events that shake space and time.
"We can't control nature, but we can control our detectors," says Lisa Barsotti, a senior research scientist at MIT who oversaw the development of the new LIGO technology, a project that originally involved research experiments at MIT led by Professor of Physics Matt Evans (PhD '02) and Professor of Astrophysics Nergis Mavalvala. The effort now includes dozens of scientists and engineers based at MIT, Caltech, and the twin LIGO observatories in Hanford, Washington, and Livingston, Louisiana.
"A project of this scale requires multiple people, from facilities to engineering and optics—basically the full extent of the LIGO Lab with important contributions from the LIGO Scientific Collaboration. It was a grand effort made even more challenging by the pandemic," Barsotti says.
"Now that we have surpassed this quantum limit, we can do a lot more astronomy," explains Lee McCuller, assistant professor of physics at Caltech and one of the leaders of the new study. "LIGO uses lasers and large mirrors to make its observations, but we are working at a level of sensitivity that means the device is affected by the quantum realm."
The results also have ramifications for future quantum technologies such as quantum computers and other microelectronics as well as for fundamental physics experiments. "We can take what we have learned from LIGO and apply it to problems that require measuring subatomic-scale distances with incredible accuracy," McCuller says.
"When NSF first invested in building the twin LIGO detectors in the late 1990s, we were enthusiastic about the potential to observe gravitational waves," says NSF Director Sethuraman Panchanathan. "Not only did these detectors make possible groundbreaking discoveries, they also unleashed the design and development of novel technologies. This is truly exemplar of the DNA of NSF—curiosity-driven explorations coupled with use-inspired innovations. Through decades of continuing investments and expansion of international partnerships, LIGO is further poised to advance rich discoveries and technological progress."
The laws of quantum physics dictate that particles, including photons, will randomly pop in and out of empty space, creating a background hiss of quantum noise that brings a level of uncertainty to LIGO's laser-based measurements. Quantum squeezing, which has roots in the late 1970s, is a method for hushing quantum noise or, more specifically, for pushing the noise from one place to another with the goal of making more precise measurements.
The term squeezing refers to the fact that light can be manipulated like a balloon animal. To make a dog or giraffe, one might pinch one section of a long balloon into a small precisely located joint. But then the other side of the balloon will swell out to a larger, less precise size. Light can similarly be squeezed to be more precise in one trait, such as its frequency, but the result is that it becomes more uncertain in another trait, such as its power. This limitation is based on a fundamental law of quantum mechanics called the uncertainty principle, which states that you cannot know both the position and momentum of objects (or the frequency and power of light) at the same time.
Since 2019, LIGO's twin detectors have been squeezing light in such a way as to improve their sensitivity to the upper frequency range of gravitational waves they detect. But, in the same way that squeezing one side of a balloon results in the expansion of the other side, squeezing light has a price. By making LIGO's measurements more precise at the high frequencies, the measurements became less precise at the lower frequencies.
"At some point, if you do more squeezing, you aren't going to gain much. We needed to prepare for what was to come next in our ability to detect gravitational waves," Barsotti explains.
Now, LIGO's new frequency-dependent optical cavities—long tubes about the length of three football fields—allow the team to squeeze light in different ways depending on the frequency of gravitational waves of interest, thereby reducing noise across the whole LIGO frequency range.
"Before, we had to choose where we wanted LIGO to be more precise," says LIGO team member Rana Adhikari, a professor of physics at Caltech. "Now we can eat our cake and have it too. We've known for a while how to write down the equations to make this work, but it was not clear that we could actually make it work until now. It's like science fiction."
Uncertainty in the Quantum Realm
Each LIGO facility is made up of two 4-kilometer-long arms connected to form an "L" shape. Laser beams travel down each arm, hit giant suspended mirrors, and then travel back to where they started. As gravitational waves sweep by Earth, they cause LIGO's arms to stretch and squeeze, pushing the laser beams out of sync. This causes the light in the two beams to interfere with each other in a specific way, revealing the presence of gravitational waves.
However, the quantum noise that lurks inside the vacuum tubes that encase LIGO's laser beams can alter the timing of the photons in the beams by minutely small amounts. McCuller likens this uncertainty in the laser light to a can of BBs. "Imagine dumping out a can full of BBs. They all hit the ground and click and clack independently. The BBs are randomly hitting the ground, and that creates a noise. The light photons are like the BBs and hit LIGO's mirrors at irregular times," he said in a Caltech interview.
The squeezing technologies that have been in place since 2019 make "the photons arrive more regularly, as if the photons are holding hands rather than traveling independently," McCuller said. The idea is to make the frequency, or timing, of the light more certain and the amplitude, or power, less certain as a way to tamp down the BB-like effects of the photons. This is accomplished with the help of specialized crystals that essentially turn one photon into a pair of two entangled, or connected, photons with lower energy. The crystals don't directly squeeze light in LIGO's laser beams; rather, they squeeze stray light in the vacuum of the LIGO tubes, and this light interacts with the laser beams to indirectly squeeze the laser light.
"The quantum nature of the light creates the problem, but quantum physics also gives us the solution," Barsotti says.
An Idea That Began Decades Ago
The concept for squeezing itself dates back to the late 1970s, beginning with theoretical studies by the late Russian physicist Vladimir Braginsky; Caltech's Kip Thorne (BS '62), Richard P. Feynman Professor of Theoretical Physics, Emeritus; and Carlton Caves (PhD '79), a former Caltech graduate student and research fellow now at the University of New Mexico. The researchers had been thinking about the limits of quantum-based measurements and communications, and this work inspired one of the first experimental demonstrations of squeezing in 1986 by H. Jeff Kimble, Caltech's William L. Valentine Professor of Physics, Emeritus. Kimble compared squeezed light to a cucumber; the certainty of the light measurements are pushed into only one direction, or feature, turning "quantum cabbages into quantum cucumbers," he wrote in an article in Caltech's Engineering & Science magazine in 1993.
In 2002, researchers began thinking about how to squeeze light in the LIGO detectors, and, in 2008, the first experimental demonstration of the technique was achieved at the 40-meter test facility at Caltech. In 2010,MIT researchers developed a preliminary design for a LIGO squeezer, which they tested at LIGO's Hanford site. Parallel work done at the GEO600 detector in Germany also convinced researchers that squeezing would work. Nine years later, in 2019, after many trials and careful teamwork, LIGO began squeezing light for the first time.
"We went through a lot of troubleshooting," says Sheila Dwyer, who has been working on the project since 2008, first as a graduate student at MIT and then as a scientist at the LIGO Hanford Observatory beginning in 2013. "Squeezing was first thought of in the late 1970s, but it took decades to get it right."
Too Much of a Good Thing
However, as noted earlier, there is a tradeoff that comes with squeezing. By moving the quantum noise out of the timing, or frequency, of the laser light, the researchers put the noise into the amplitude, or power, of the laser light. The more powerful laser beams then push LIGO's heavy mirrors around causing a rumbling of unwanted noise corresponding to lower frequencies of gravitational waves. These rumbles mask the detectors' ability to sense low-frequency gravitational waves.
"Even though we are using squeezing to put order into our system, reducing the chaos, it doesn't mean we are winning everywhere," says Dhruva Ganapathy, a graduate student at MIT and one of four co-lead authors of the new study. "We are still bound by the laws of physics." The other three lead authors of the study are MIT graduate student Wenxuan Jia, LIGO Livingston postdoctoral scholar Masayuki Nakano, and MIT postdoctoral scholar Victoria Xu.
Unfortunately, this troublesome rumbling becomes even more of a problem when the LIGO team turns up the power on its lasers. "Both squeezing and the act of turning up the power improve our quantum-sensing precision to the point where we are impacted by quantum uncertainty," McCuller says. "Both cause more pushing of photons, which leads to the rumbling of the mirrors. Laser power simply adds more photons, while squeezing makes them more clumpy and thus rumbly."
A Win-Win
The solution is to squeeze light in one way for high frequencies of gravitational waves and another way for low frequencies. It's like going back and forth between squeezing a balloon from the top and bottom and from the sides.
This is accomplished by LIGO's new frequency-dependent squeezing cavity, which controls the relative phases of the light waves in such a way that the researchers can selectively move the quantum noise into different features of light (phase or amplitude) depending on the frequency range of gravitational waves.
"It is true that we are doing this really cool quantum thing, but the real reason for this is that it's the simplest way to improve LIGO's sensitivity," Ganapathy says. "Otherwise, we would have to turn up the laser, which has its own problems, or we would have to greatly increase the sizes of the mirrors, which would be expensive."
LIGO's partner observatory, Virgo, will likely also use frequency-dependent squeezing technology within the current run, which will continue until roughly the end of 2024. Next-generation larger gravitational-wave detectors, such as the planned ground-based Cosmic Explorer, will also reap the benefits of squeezed light.
With its new frequency-dependent squeezing cavity, LIGO can now detect even more black hole and neutron star collisions. Ganapathy says he's most excited about catching more neutron star smashups. "With more detections, we can watch the neutron stars rip each other apart and learn more about what's inside."
"We are finally taking advantage of our gravitational universe," Barsotti says. "In the future, we can improve our sensitivity even more. I would like to see how far we can push it."
The Physical Review X study is titled "Broadband quantum enhancement of the LIGO detectors with frequency-dependent squeezing." Many additional researchers contributed to the development of the squeezing and frequency-dependent squeezing work, including Mike Zucker of MIT and GariLynn Billingsley of Caltech, the leads of the "Advanced LIGO Plus" upgrades that includes the frequency-dependent squeezing cavity; Daniel Sigg of LIGO Hanford Observatory; Adam Mullavey of LIGO Livingston Laboratory; and David McClelland's group from the Australian National University.
The LIGO–Virgo–KAGRA Collaboration operates a network of gravitational-wave detectors in the United States, Italy, and Japan. LIGO Laboratory is operated by Caltech and MIT, and is funded by the NSF with contributions to the Advanced LIGO detectors from Germany (Max Planck Society), the U.K. (Science and Technology Facilities Council), and Australia (Australian Research Council). Virgo is managed by the European Gravitational Observatory (EGO) and is funded by the Centre national de la recherche scientifique (CNRS) in France, the Istituto Nazionale di Fisica Nucleare (INFN) in Italy, and the National Institute for Subatomic Physics (Nikhef) in the Netherlands. KAGRA is hosted by the Institute for Cosmic Ray Research (ICRR) at the University of Tokyo and co-hosted by the National Astronomical Observatory of Japan (NAOJ) and the High Energy Accelerator Research Organization (KEK).
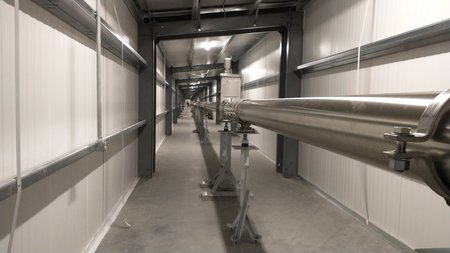
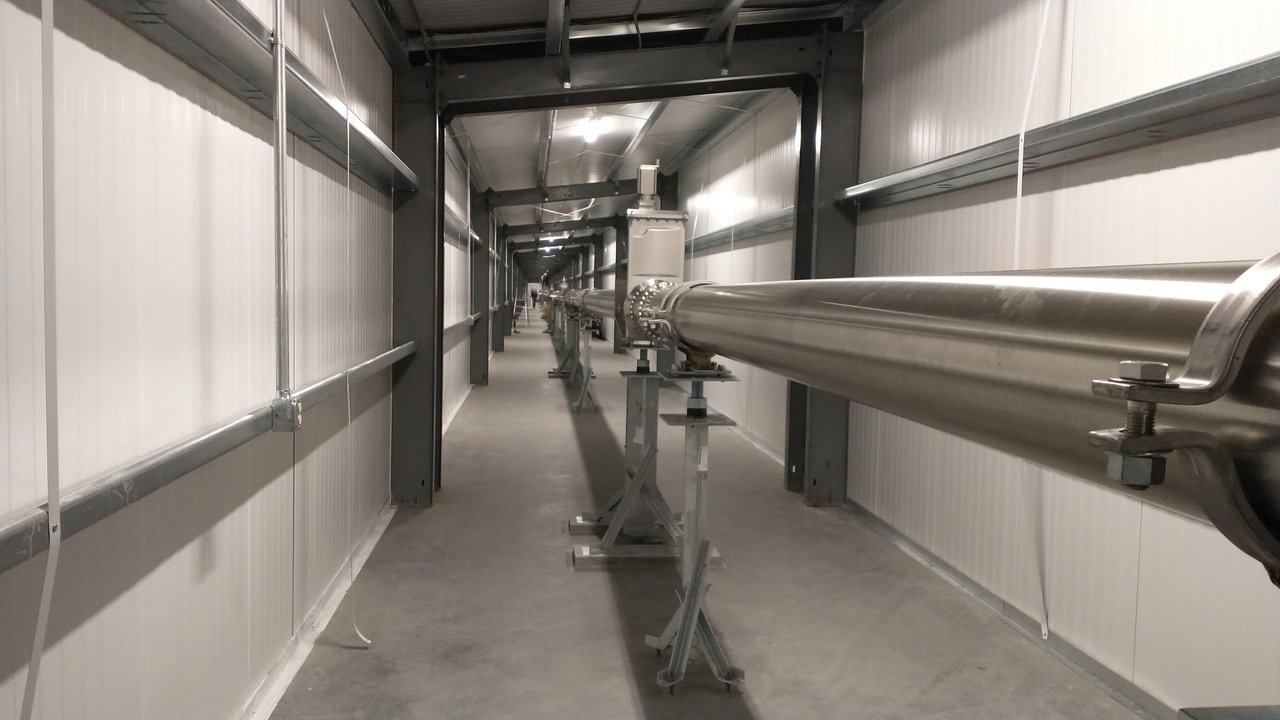
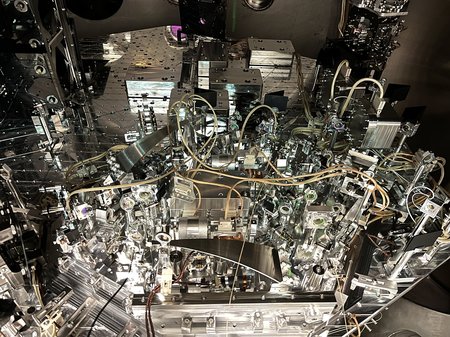
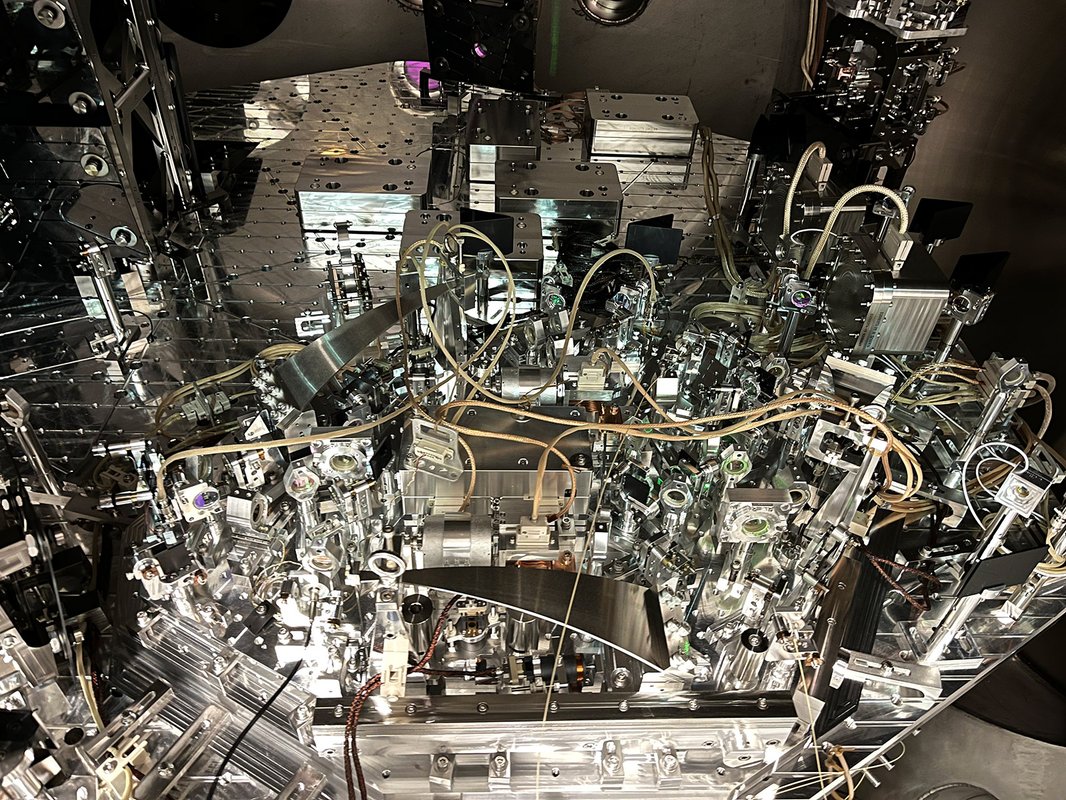
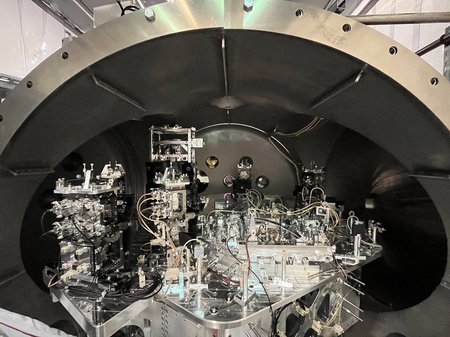
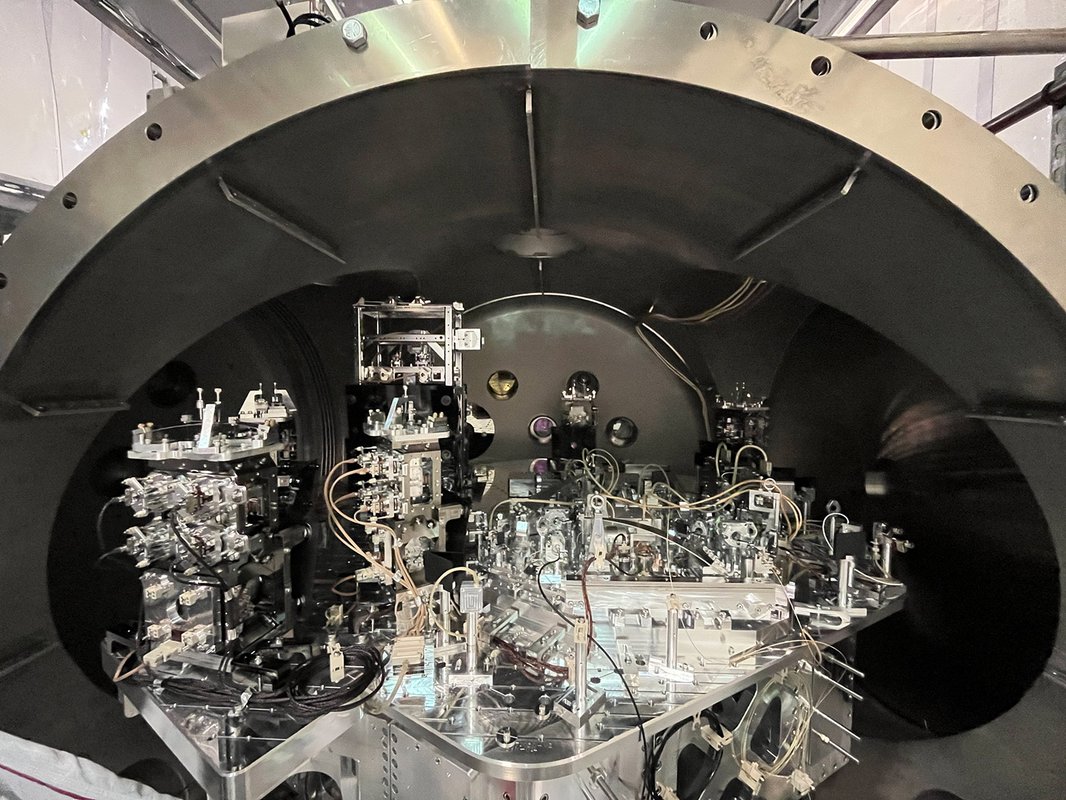
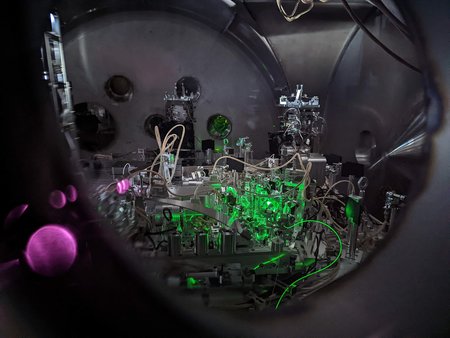
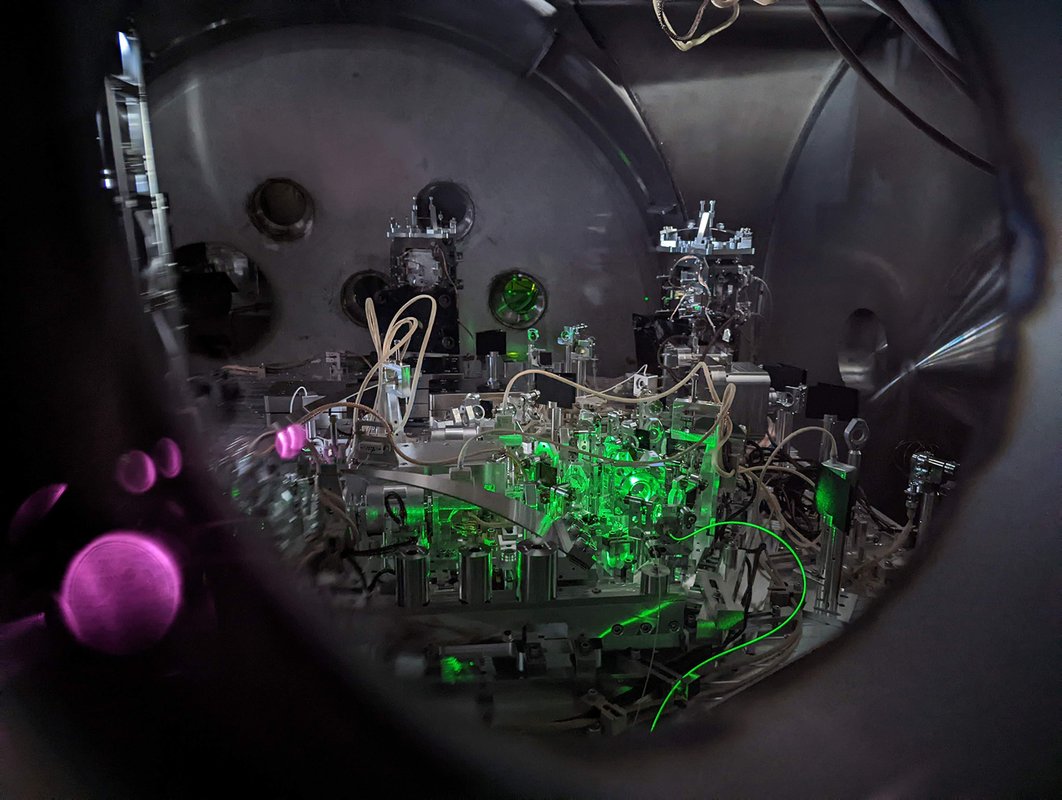